10 Transportation and Climate Change Mitigation: A Life-Cycle Assessment Perspective
Chapter Overview
This chapter is divided into three sections. The first examines the significance of transportation for domestic and global GHG (Greenhouse Gas) emissions in the United States and provides a global perspective of how this contribution varies in other countries. The second uses life-cycle assessment as a comprehensive methodological approach to estimate GHG emissions associated with constructing and using transportation systems. Next, the chapter examines the transportation sector’s contribution to national and global GHG emissions.
Learning Objectives
- Explain the benefits of Life Cycle Assessment (LCA) as a methodological approach to measuring the contribution to GHG emissions and air pollutants by transportation.
- Estimate the significance of transportation, specifically private transport, to domestic and global GHG emissions.
- Compare how the share of transportation-related GHG emissions differs between developed and developing countries, identifying the factors that drive these differences.
- Contrast how driving, public transit, and non-motorized transit use rates vary among U.S. cities.
- Estimate the carbon footprint associated with commuting patterns across various U.S. cities.
CITIES, Transportation, and Climate Change Mitigation
Large cities are the most significant contributors to GHG emissions because they concentrate the largest populations and activities. As mentioned in Chapter 1, U.S. cities are among the top emitters of GHG emissions associated with transportation partly due to urban form-related variables, including low-population density, fewer public transportation options, and difficult access to public transit. On the other hand, European cities are more compact and have various mobility options. As a result, low-density communities, like those in the United States, where most individuals commute between their places of origin and destinations alone in private vehicles, have greater transportation energy consumption. Cities in the Global South tend to have even denser populations and greater access to public transportation. Therefore, these cities consume less energy for transportation than developed-world cities do. In addition, people typically walk and take public transportation in the Global South (Guerra et al., 2020). As a result, Global South cities tend to contribute less to GHG emissions and use less energy than most developed-world cities, mainly low-density and automobile-dependent ones.
As discussed in Chapter 1, the contribution of urban residents to GHG emissions largely depends on several factors, including population density and the proximity of residences to job locations. For example, residents of compact urban areas in central locations contribute significantly less than residents in the suburbs, as exemplified by Toronto, Canada, in the study conducted by Norman et al. (2006). Therefore, GHG assessment requires a thorough understanding of urban densities and how commuters use motorized vehicles, transit, and non-motorized transportation systems to get to places.
Climate change mitigation of greenhouse gas emissions is one of cities’ and countries’ most critical environmental and social challenges. Cities are essential in climate change mitigation because they contribute to 70% of global GHG emissions, projected to reach 76% by 2030 (Chambers & Nakicenovic, 2008). As mentioned in Chapter 2, another significant issue that imperils commuters worldwide and transportation systems is the connection between transportation and climate change. Extreme climatic events threaten vehicles’ infrastructure, procedures, and urban mobility, even though transportation energy usage is the primary source of GHG emissions in U.S. cities (Jochem et al., 2016; Moretti & Loprencipe, 2018).
However, the contributions of cities in the North and the South significantly vary. Developed cities in the Global North are the largest emitters of GHG emissions due to the large concentration of population, activities, and unsustainable energy-use practices. Table 10.1 illustrates how the contributions of countries and cities differ. Large cities with high population densities allow efficient and widely used transportation systems while enabling non-motorized transportation and economies of scale. For instance, Buenos Aires contributes less than smaller Argentinian cities, such as Avellaneda, and its contribution is less than the national average in Argentina. Likewise, U.S. cities in the Northeast, like Philadelphia, which tend to have dense urban centers and relatively good transportation, contribute less than car-dependent cities, such as Austin, Texas. European countries illustrate how tight urban areas moderate the contribution of GHG emissions. The national average is smaller in countries like Norway and France than in their capital cities.
Interestingly, China’s national average GHG emissions (3.25 TCO2 per capita) are smaller than big, modern cities like Shanghai (12.6 TCO2e). Shanghai could be among the world’s top 40 countries in terms of GHG emissions if it were an independent country. China’s large population densities and the low energy consumption of residents in urban and rural areas may explain China’s low per-capita contribution. However, because China is still rapidly urbanizing, its contribution to GHG emissions is growing, and now it is the most significant contributor if we consider the total emissions of the entire population (Hoornweg, Sugar, & Trejos Gómez, 2011).
The contribution of cities and countries to GHG emissions highly depends on how economies use energy and resources. To better mitigate GHG emissions, researchers should carefully understand how different economic sectors, such as buildings and transportation, use energy and resources. As mentioned in Chapter 2, the fastest way to reduce car driving and thus mitigate greenhouse gas emissions in the transportation sector is to improve commuters’ access to multimodal transit, such as the interconnection of the subway, buses, and biking infrastructure. Also, land-use planning can help reduce car driving through enhanced community design, walkable streets, and diversified land uses. Fostering greater densities, above 40 people per hectare, mixed-use complexes integrating residential and commercial land use, and accessible linkages to public transportation hubs are alternate growth patterns that might enable sustainable cities. Sustainable mobility demands excellent land-use planning and transportation integration to reduce commuting times and facilitate multimodal shifts. A population with the proper density can improve quality of life by reducing reliance on cars.
Another factor is the extent of population densities and peoples’ income and energy-use practices. Low-income residents use less energy and contribute less to emissions than wealthier families. However, cities may influence energy consumption. For instance, despite the average income in Denver ($65,400) being lower than that of the New York Metro area ($73,000), the average energy consumption of an NYC resident was nearly half that of a Denver resident. This discrepancy is mainly attributable to higher urban density in New York and lower dependency on private cars for daily commutes. This situation reveals that cities offer the most significant opportunities to reduce the energy consumption of buildings, infrastructure, and residents’ transportation practices while supporting social justice. In addition, cities matter for climate change because low and emerging countries and cities in the Global South are perhaps the most vulnerable to extreme climate events, even though they tend to contribute less to GHG emissions (Darmstadter, 2010).
Also, GHG emissions vary significantly at the neighborhood level. For example, a study by Vandeweghe and Kennedy (2007), which analyzed the consumption-based and production-based transport emissions by census tracts for Toronto, found that residents in the city core produced 6.42 tCO2e per capita compared to 7.74 tCO2e per capita for residents in the surrounding suburbs. These suburbs were mainly affluent neighborhoods with high auto dependency and old, inefficient homes. Additionally, they found that the lowest emission was 1.31 tCO2 per capita for a dense inner-city area with good access to public transportation. Also, the highest emissions were 13.02 per capita in distant suburbs.
Cities and regions are the focal points of climate change mitigation due to the high concentration of emissions in these areas. Still, they provide a unique opportunity to mitigate per-capita GHG emissions through local policy tools, which can directly and promptly affect residents’ travel behavior and energy-use practices (Hoornweg, Sugar, & Trejos Gómez, 2011). As explored in previous chapters, some cities have used comprehensive transportation and land-use planning policy approaches and have combined several interventions, including parking pricing, congestion pricing, and dense and integrated land developments. These cities include Singapore, Stockholm, San Francisco, and London. They all have influenced commuter travel behavior and support sustainable communities. The initial step for devising policy tools to address climate change and GHG emissions is to explore the sources within each urban settlement. For example, a highly industrialized city may produce high production-based emissions, while a knowledge-based economy in a city with public transit may produce significantly lower emissions.
Country GHG emissions (tCO2e/capita) |
blank cell |
---|---|
Argentina | 7.64 |
Buenos Aires | 3.83 |
Avellaneda | 6.53 |
Norway | 11.69 |
Oslo | 3.5 |
China | 3.4 |
Shanghai | 11.7 |
India | 1.33 |
Delhi | 1.5 |
France | 8.68 |
Paris | 5.2 |
Italy | 9.31 |
Bologna | 11.1 |
Czech Republic | 14.59 |
Prague | 9.4 |
USA | 23.59 |
Austin | 15.57 |
Philadelphia | 11.1 |
Los Angeles | 13 |
Mexico | 5.53 |
Mexico City (City) | 4.25 |
Mexico City (Metro Area) | 2.84 |
Adapted from “Cities and greenhouse gas emissions: Moving forward” by Hoornweg, D., Sugar, L., & Trejos Gómez, C. L., 2011, Environment and Urbanization, 23(1), p.211, Copyright 2011 by International Institute for Environment and Development (IIED)
LIFE-Cycle Assessment in Transportation
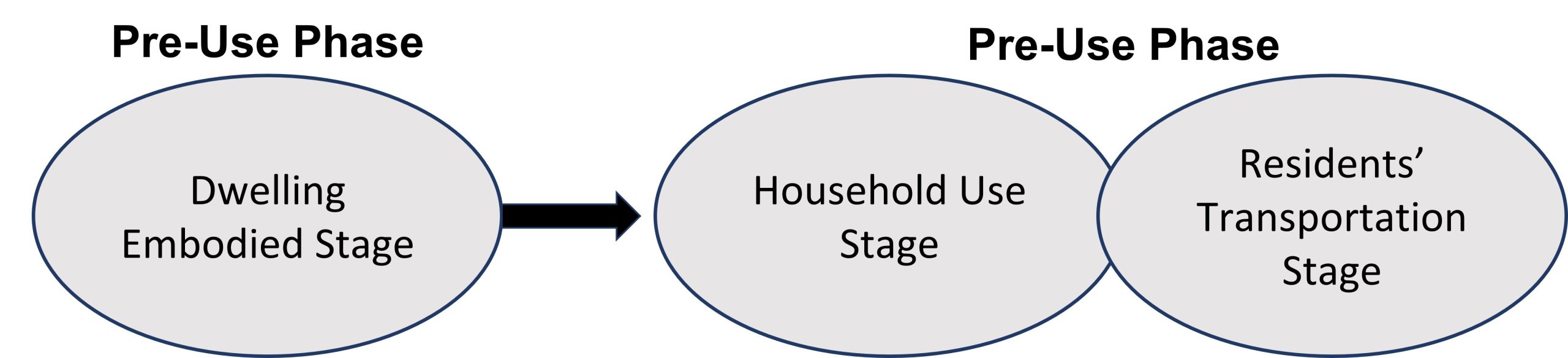
Life-cycle assessment (LCA) is an Environmental Impact Assessment method for thoroughly assessing energy use and greenhouse gas emissions associated with buildings, infrastructure, and technological innovations (Fuller & Crawford, 2011; Ramesh et al., 2010; Stephan et al., 2011). Data about systems, technologies, and infrastructure can be separated into two main life-cycle phases: embodied and operating. The embodied phase (also called the construction phase) includes the use of energy, resources, and materials that result from the manufacture of building materials, the transportation of materials to the construction site, the construction of infrastructure and technological innovations, and demolitions, as well as the disposal and recycling of materials (see Figure 10.1). In transportation, the operating phase includes using energy, resources, and materials that result from using infrastructure or transportation, which means commuters use them during a trip.
LCAs of residential land-use Planning in developed-world Cities
This section analyses significant LCAs (Life Cycle Assessments) of residential land use in established cities to determine the energy usage in residential land use and transportation. In Toronto, low-density suburban development is 2-2.5 times more energy intensive in terms of energy use and GHG emissions than high-density, urban core development, according to Norman and colleagues’ full life-cycle assessment, which included embodied energy, operating energy, and residents’ transportation energy (Norman et al., 2006). Additionally, they discovered that inhabitants’ fuel use is influenced by transportation energy since suburban development boosts automobile utilization (Norman et al., 2006). An in-depth analysis of the energy use of typical single-family homes in Belgium was performed by Stephen et al. in 2011. They discovered that home energy usage was primarily driven by transportation, which accounted for 34-51% of the whole life-cycle energy consumption, and domestic appliance operation, which accounted for 24% of the total life-cycle energy consumption (Stephan et al., 2011a). According to the LCA findings of Norman et al. (2006) and Stephan et al. (2011a), transportation energy dominates residential energy usage in the total life-cycle of energy when taken into account. Accordingly, it can be deduced from prior GHG evaluations that urban density and the accessibility of housing projects to transportation networks affect how much fossil fuels commuters consume to go to and from places of residence to areas with plenty of jobs (Fuller & Crawford, 2011; Lee & Lee, 2014; Stephan et al., 2011a).
i. Methodological debates
Methodological debates regarding the use of LCAs in residential land use analysis focus on the scope of life-cycle assessment. In other words, LCAs of residential land use differ in the extent to which life-cycle phases are analyzed. Although transportation energy accounts for the highest energy use in residential developments, most LCAs completely disregard it (Ramesh et al., 2010). Most GHG assessments in the housing sector examine dwelling embodied energy or operating energy use but ignore residents’ transportation energy use (Verbeeck & Hens, 2010). Stephen et al. (2011a) argue that a more holistic approach to assessing energy use in dwellings is indispensable to effectively mitigating GHG emissions in residential land-use planning. They suggest widening the typical scope of analysis, currently restricted to household energy use, to also account for dwelling embodied energy, including incremental housing construction practices over time (Verbeeck & Hens, 2010), but more importantly, to account for residents’ transportation energy (see Figure 10.2) (Stephan et al., 2011a).
ii. Limitations of life-cycle assessment
This section discusses some of the limitations of the life-cycle review of residential land use as an environmental impact assessment method developed and implemented in the context of developed cities. Also, this section considers that LCA has been informed solely by quantitative approaches that cannot explain the complex nature and extent of energy use in residential land use. In doing so, the section explores the possibility of informing LCA with the qualitative assessment that may better explain the complexity of energy use in land-use residential planning.
Most GHG assessments in the housing sector have been conducted in developed-world cities, particularly in North America and Europe (Ramesh et al., 2010). Therefore, it can be argued that there is a poor understanding of the contribution to GHG emissions of housing units in these cities. This situation suggests that a life-cycle assessment of residential land use in developing global cities could better understand energy use characteristics and extent and compare different types of residential development (Stephan et al., 2011a).
The second methodological debate over previous LCAs in the residential sector suggests that for life-cycle assessment to be practical, it must include the complete life cycle of the housing unit as an interconnected system incorporating sub-systems. An incomplete review of the housing unit’s life cycle can lead to an incomplete and misleading understanding of related energy use. In the case of residential land use, the housing unit can be considered a system that includes dwelling units, infrastructural elements, people using energy in dwelling units, and people conducting everyday transportation activities to and from those units (Stephan et al., 2011a).
Third, life-cycle assessment overly relies on quantitative methods, particularly statistical analysis and surveys. LCA has been widely applied in engineering to compare the environmental impacts of well-defined systems, such as conventional cars and electric automobiles (Matsuhashi et al., 2000). Therefore, engineers should use planning research methods to complement the current quantitative methods in LCAs. These planning methods include surveys, in-depth interviews, and the collection of oral stories of residents to reveal the complex nature of energy use and the obstacles and opportunities to supporting climate change mitigation and, at the same time, keeping the living conditions.
Examples of LCA in Transportation
This section examines past LCAs, exploring how the built environment and transportation infrastructure influence GHG emissions. Integrated transportation and land use (ITLU) life-cycle assessment can yield information about behavioral changes associated with urban transformation, informing decisions about urban infrastructure policies regarding environmental outcomes (Chester et al., 2013). ITLU-LCAs assess the ecological impacts of residential land use and transportation associated with residential and commercial travel and identify socially and economically sensitive land-use configurations that may support climate change mitigation and housing affordability.
Chester et al. (2013) examined the environmental implications of the Phoenix light rail infrastructure. Changes in energy use, GHG emissions, and other pollutants have been monitored and assessed for over 60 years due to investment in transit-oriented developments (TOD). This study selects different types of neighborhoods (low-income with vacant lots, middle-income and detached housing units, and areas near downtown) for analysis. One of the critical factors for TOD development is the 0.5-mile walking distance from the station (Cervero, 2004); thus, sites meeting this criterion were selected. This study considered four development scenarios, including (i) low-density development on vacant and surface parking by constructing single homes, (ii) high-density residential development along with multistory commercial spaces, (iii) new construction on vacant lands and parking surfaces and the reuse of existing buildings, and (iv) demolition of all residential, commercial, and industrial buildings in the lowest quartile of market value and replacing them with new high-density mixed-use buildings. This study revealed that infill development with the availability of public transit amenities has a great potential to reduce life-cycle energy consumption and GHG emissions. Moreover, social benefits were anticipated with more intense and denser development.
This comprehensive LCA assessment found that transportation and building are responsible for 22-51% and 22-44% of energy consumption and GHG emissions, respectively. Commercial activities have 1.9-3.3 times more significant effects than residential ones. 31% saving in life-cycle energy consumption can be achieved in scenario 1, and in the other three scenarios, this saving can be as high as 42%. Analyzing scenario 1, which only focuses on infill development, shows that locating households near transit stations rather than sprawled neighborhoods produces an opportunity to shift from private cars to mass transit and shorter travel, resulting in significant savings in life-cycle energy consumption. Based on scenario 2, substituting a single home with a mixed-use high density will increase these savings by 38. For the construction phase, high-density residential development produces 1.5-3 times more energy impacts per dwelling unit in the construction phase but generates 10% less energy use in 60 life-cycle spans. Exploring the four scenarios for commercial land use shows that locating commercial establishments closer to the core with access to public transit reduces automobile use for shopping trips, reduces the distance of average shopping trips, and induces shopping trips via public transit even from outside the area. It is interesting that in scenarios 2-4, nonresidents’ energy use reductions are 2.2-4.4 larger than reductions from residents inside the TOD area. Such findings suggest that implementing environmental policies and programs can benefit city-wide. In general, it can be stated that LCA can yield various kinds of conclusions for different phases, which is crucial for policymakers in designing programs. Built environment and land-use planning significantly influence environmental issues like energy savings and GHG emissions. However, understanding the interplay between various factors, from the building phase, electricity provision, travel behavior, travel costs, etc., is crucial for long-term planning and design (Chester et al., 2013).
The induced impacts of the built environment as a result of the interplay between individual building construction and urban scale is another subject that this chapter intends to unpack. The energy consumption of the building and transportation sectors will likely increase between 20% and 44% from 2009 to 2035 (Birol, 2010). Induced impacts in this subject refer to interactions between individual buildings and their surrounding context. In a study in Munich, Germany, Anderson, Wulfhorst, and Lang (2015) examined the induced impacts associated with buildings and the urban context via transportation measures using a life-cycle assessment perspective. These measures include the transportation use phase and the embodied effects of transportation infrastructure and vehicles. Munich’s rich transportation network supports mass transit, walking and biking, and automobiles. For this analysis, three types of development in Munich were selected: city center, city periphery, and district locations (outside Munich).
A preliminary analysis of the dataset used in this study showed that Munich residents have a modal split of 27% driving private cars, 10% riding personal vehicles, 21% public transit, 14% biking, and 28% walking. This share differs in the city’s outskirts, where 17% drive a private car, 15% ride personal vehicles, 7% ride public transit, 11% cycle, and 20% walk. This study found that the city center has the lowest total emission, and the periphery area and the city are 18.5% and 33.5% higher than the city center. Analyzing operation costs of transportation shows that, on average have lower travel distance and CO2e emissions. However, since Munich has a well-developed public transit system that covers almost the entire city, a transparent decreasing gradient for travel distance cannot be seen from the city center to the periphery. This situation again highlights the importance of public transit in realizing environmentally friendly goals. The road network has the lowest emissions from embodied (construction and materials) transportation impacts.
In contrast, public transit infrastructure has much higher embodied impacts because constructing tunnels and bridges significantly requires high amounts of materials and energy. However, private cars are the largest emitters of GHG emissions partly because of the considerably shorter life span of vehicles compared to public transit vehicles. Also, the high number of cars and long commutes increase the embodied costs of roads and the fossil fuels consumed in trips. Furthermore, the production of cars has significant embodied impacts, which increase the total share of GHG emissions associated with private cars (Anderson, Wulfhorst, & Lang, 2015). Overall, this section reveals the importance of comprehensively examining the impacts of transportation systems, from the extraction of materials to the construction and use of vehicles. Another essential element is the comparative analysis of how commuters in different locations within metropolitan areas and cities, urban locations versus suburban and peripheral zones, contribute to GHG emissions.
Conclusion
Overall, this chapter reveals the importance of a comprehensive examination of the impacts of transportation systems, from the extraction of materials to the construction and use of vehicles. Another essential element is the comparative analysis of commuters’ contributions in different locations within metropolitan areas and cities, suburban and peripheral locations. Finally, the place of residential development within urban areas determines how residents use transportation energy for daily commutes. Those in the suburbs tend to contribute less because they travel longer distances in automobiles.
Glossary
- Carbon footprint is the volume of carbon dioxide and other carbon compounds released from a specific individual, organization, or other entity using fossil fuels (National Park Service, n.d.).
- Climate change mitigation implies preventing or limiting the amount of greenhouse gas emissions released into the atmosphere to stop the world from warming to more extreme levels (World Wildlife Fund, n.d.).
- Environmental impact assessment Before deciding to take the suggested action, a strategy, policy, program, or actual project is evaluated for its potential impact on the environment—the phase in this situation. (Climate Change Scavenger Hunt – National Park Service, https://www.nps.gov/teachers/classrooms/climate-change-scavenger-hunt.htm)
References
Anderson, J. E., Wulfhorst, G., & Lang, W. (2015). Comprehensive analysis of the built environment through the introduction of induced impacts via transportation: Detailed case study for the urban region of Munich, Germany. Transportation Research Record, 2500(1), 67–74. https://doi.org/10.3141/2500-08
Birol, F. (2010). World energy outlook2010. International Energy Agency. https://www.iea.org/reports/world-energy-outlook-2010
Cervero, R. (2004). Transit-oriented development in the United States: Experiences, challenges, and prospects. Vol. 102. Transportation Research Board. https://www.trb.org/Publications/Blurbs/154989.aspx
Chambers, A., & Nakicenovic, N. (2008, November 1). World energy outlook 2008; IEA. https://doi.org/10.1787/weo-2002-en
Chester, M. V., Nahlik, M. J., Fraser, A. M., Kimball, M. A., & Garikapati, V. M. (2013). Integrating life-cycle environmental and economic assessment with transportation and land use planning. Environmental Science & Technology, 47(21), 12020–28. https://doi.org/10.1021/es402985g
Bierbaum, R. M., Fay, M., & Ross-Larson, B. (2010). World development report 2010: development and climate change (English). World Bank Group. 52(2). 53077. http://documents.worldbank.org/curated/en/201001468159913657/World-development-report-2010-development-and-climate-change
Darmstadter, Joel. “The Prospective Role of Unconventional Liquid Fuels.” Resources for (2010). https://media.rff.org/documents/RFF-BCK-Darmstadter-AltLiquidFuels.pdf
Guerra, E., Li, S., & Reyes, A. (2020). How do low-income commuters get to work in U.S. and Mexican cities? A comparative empirical assessment. Urban Studies. 59(1), 75–96. https://doi.org/10.1177/0042098020965442
Hoornweg, D., Sugar, L., & Trejos Gómez, C. L. (2011). Cities and greenhouse gas emissions: Moving forward. Environment and Urbanization, 23(1), 207–27. https://doi.org/10.1177/0956247810392270
Jochem, P., Rothengatter, W., & Schade, W. (2016). Climate change and transport. Transportation Research Part D: Transport and Environment, 45, 1–3. https://doi.org/10.1016/j.trd.2016.03.001
Matsuhashi, R., Kudoh, Y., Yoshida, Y., Ishitani, H., Yoshioka, M., & Yoshioka, K. (2000). Life cycle of CO 2-emissions from electric vehicles and gasoline vehicles utilizing a process-relational model. The International Journal of Life Cycle Assessment, 5, 306-312.
Moretti, L., & Loprencipe, G. (2018). Climate change and transport infrastructures: State of the art. Sustainability, 10(11), 4098. Publicly Available Content Database; SciTech Premium Collection. https://doi.org/10.3390/su10114098
Norman, J., MacLean, H. L., & Kennedy, C. A. (2006). Comparing high and low residential density: Life-cycle analysis of energy use and greenhouse gas emissions. Journal of Urban Planning and Development, 132(1), 10–21. https://doi.org/10.1061/(ASCE)0733-9488(2006)132:1(10)
Ramesh, T., Prakash, R., & Shukla, K. K. (2010). Life cycle energy analysis of buildings: An overview. Energy and buildings, 42(10), 1592-1600.
Stephan, A., Crawford, R. H., & De Myttenaere, K. (2013). A comprehensive assessment of the life cycle energy demand of passive houses. Applied energy, 112, 23-34. https://doi.org/10.1016/j.apenergy.2013.05.076
VandeWeghe, J. R., & Kennedy, C. (2007). A spatial analysis of residential greenhouse gas emissions in the Toronto Census Metropolitan Area. Journal of Industrial Ecology, 11(2), 133–44. https://doi.org/10.1162/jie.2007.1220
are the different gases released into the planet's atmosphere, particularly carbon dioxide, which contribute to the greenhouse effect.
is a methodology for assessing environmental impacts associated with all the life cycle stages of a commercial product, process, or service. (Khadour, 2011)
is the volume of carbon dioxide and other carbon compounds released from a specific individual, organization, or other entity using fossil fuels. (National Park Service, n.d.)
implies preventing or limiting the amount of greenhouse gas emissions released into the atmosphere to stop the world from warming to more extreme levels. (World Wildlife Fund, n.d.)
is the regions of Latin America, Asia, Africa, and Oceania
entails restricting or blocking the release of greenhouse gas emissions into the atmosphere to prevent the earth from warming to more extreme levels. (Investopedia, 2012)
Before deciding to take the suggested action, a strategy, policy, program, or actual project is evaluated for its potential impact on the environment—the phase in this situation.(Climate Change Scavenger Hunt - National Park Service. https://www.nps.gov/teachers/classrooms/climate-change-scavenger-hunt.htm)