Chapter 6: Introduction to Zero Emissions Vehicles
Chapter Overview
In this chapter, we discuss the concept of zero (almost no) emissions (ZEV) vehicles to low emissions vehicles. Often termed as alternative fuel vehicles, these vehicles are hailed as a more sustainable counterpart of traditional gasoline and diesel operated vehicles. The alternatives that would be discussed are battery electric vehicles, hybrid vehicles, fuel cell vehicles, hydrogen vehicles, compressed natural gas vehicles, and liquid petroleum gas vehicles. We will discuss all form factors and vocation types as it pertains to movement of people and goods – from scooters to mass transit, from last and first mile delivery to continental trucking.
Chapter Topics
- Role of Combustion in Emissions Generation
- Zero Emissions Power Generation for Transportation
- Electricity and Hydrogen as Zero Carbon Fuels for Transportation
- A California Case Study for Zero Emissions Buses
- Heavy Duty Vehicle Electrification
- California ZEV Market and Incentives Programs
Learning Objectives
At the end of the chapter, the reader should be able to do the following:
- Select appropriate ZEV technology to sustainably meet any specific travel needs.
- Evaluate the infrastructure and investment needs for different ZEV technologies.
- Reflect on the current ZEV adoption and prescribe public and private financial mechanisms to promote adoption of these vehicles.
The Role of Combustion in Emissions Generation
Combustion is the principal technology that powers the energy economy. Simply stated, combustion is at the heart of our everyday lives, from the provision of electricity to our home and place of work, to the automobiles we drive, to the propulsion of jet aircraft we fly. Combustion is also the principal source of the environmental impact we experience, from climate change to degraded urban air quality. The following four principal forces are driving the paradigm shifts from our dependency on combustion to alternative technologies for the generation of electricity and powering of vehicles:
- Degraded urban air quality (1943): The first evidence of persistently degraded urban air quality in the United States was chronicled in the Los Angeles Times, describing a tenacious haze that seemed to irritate eyes and cause many to cough (Figure 6.1). Today, urban regions throughout the world (for example, in India, China) are affected by degraded air quality.
Figure 6.1: Los Angeles 1943: Degraded Unraban Air Quality by Ramanathan et al is licensed under CC BY-NC-SA 4.0 - Finite petroleum resources (1980s): Automobile companies recognized that petroleum was finite and demand may outweigh discovery in the next millennium.
- Climate change (1990s): The world recognized that anthropogenic sources may be affecting the climate, leading to the signing of the UN Framework Convention on Climate Change in 1992.
- Fuel independence (2001): The assault on the World Trade Center enhanced the urgency to reduce US dependence on foreign sources of petroleum.
Depending on the type of engine, either air is compressed to a high pressure and fuel is added, or a fuel-air mixture is compressed to a high pressure. In both cases, the fuel-air mixture is then ignited, initiating a combustion process (essentially “burning” the fuel-air mixture) that transforms the energy bound in the fuel (for example, gasoline) to high-temperature gas (thermal energy). The high-pressure, high-temperature gas then pushes on a piston (to power the transmission in a traditional gasoline vehicle, or generate electricity in a gasoline hybrid vehicle) or expands through a turbine (to generate electricity for the home and business). From this process, depicted in Figure 6.2, you can intuitively deduce that (1) the efficiency (the percentage of energy bound in the fuel that is transformed to useful power) will be limited by the friction associated with all of the mechanical steps, and (2) criteria pollutants will be formed because of combustion chemistry and emitted in the exhaust.
When you consider the role of combustion in everyday life, the examples seem limitless (for example, cooking; heating water; space heating; generating electricity; propelling aircraft and rockets; and powering automobiles, buses, trucks, locomotives, and ships). Simply stated, combustion is interwoven into the fabric of both the quality of life and the economics of the world’s markets.
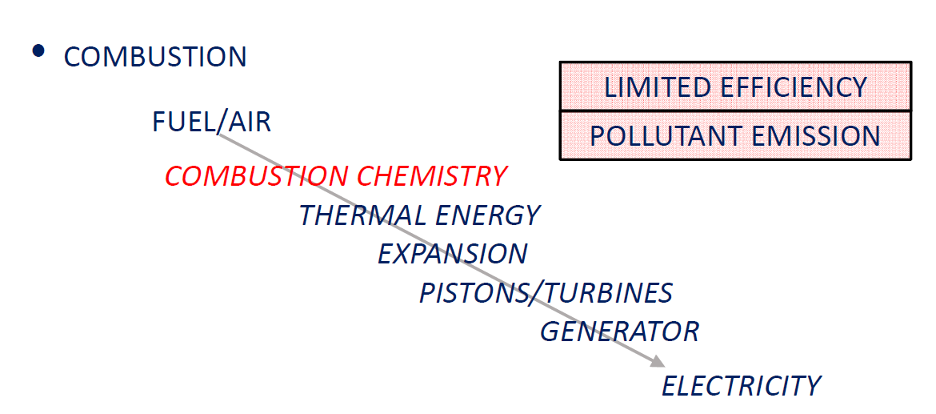
In Figure 6.3, the relationship between combustion and the environment is illustrated. Fuel and air are injected into a chamber, ignited to liberate the energy bound in the fuel into thermal energy, and expanded to produce a useful product.
Unfortunately, combustion has an exhaust as a by-product composed of criteria pollutants that degrade urban air quality (affecting the public health) and carbon dioxide (affecting the world’s climate). Notably, the amount of criteria pollutant mass in the exhaust is minuscule and was historically ignored until the first consequences to public health in modern times surfaced in 1943 (Los Angeles) and 1952 (London).[1] It is as if Nature incorporated environmental impacts in the combustion of fossil fuels to counsel the world’s population that combustion is not sustainable.
Why is it that such a minuscule emission of a few chemical criteria relatively modest emission of CO2 affects the world’s climate? Consider that the atmosphere is evenly distributed in a thin layer around the Earth, barely 10 miles in depth. In Figure 6.3, the purple sphere in the image represents the volume of all the air if it were gathered together, relative to the volume of the Earth. The image conveys the surprisingly small air resource upon which life on Earth depends, and the relatively small volume of air into which products of combustion are injected. Within this small volume, CO2 and other greenhouse gases (GHGs) accrue to affect climate, and secondary criteria pollutants are formed and primary criteria pollutants amass to degrade urban air quality. As noted in Figure 6.3, combustion is responsible for over 90% of the world’s emission of CO2 and criteria pollutants.
In addition to contaminating the air resource with CO2 and criteria pollutants, the combustion process has an impact not widely recognized: namely the consumption of oxygen from the air. For every tankful of gasoline in your car, a ton of air (2,000 pounds) passes through your engine, and 400 pounds of oxygen are consumed. Given the finite resource of oxygen in the atmosphere, this is sobering. While Nature appears to be replenishing the oxygen removed to date, an increasing demand for oxygen could lead to an additional point of environmental stress. Fortuitously, the evolving transition from a classic “combustion-dominant construct” to a “renewable-dominant construct” will, in parallel with reducing the emission of CO2 and criteria pollutants, serve to mitigate the likelihood of this environmental stress.
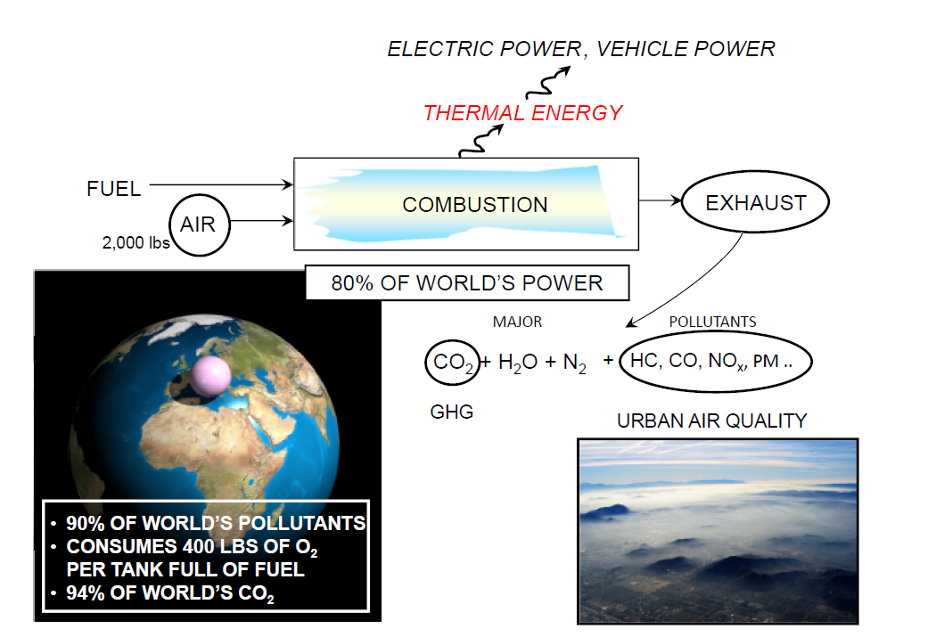
An Alternative to Combustion
Because combustion emits carbon dioxide and criteria pollutants as unavoidable by-products, an alternative to combustion that can operate (1) more efficiently than combustion (thereby reducing CO2 per megawatt hour), (2) with a zero-carbon fuel (thereby emitting no CO2), and (3) without the emission of criteria pollutants would be preferred.
An emerging alternative to combustion is fuel cell technology (Figure 6.4), which converts fuel and air to electricity in a single step. Intuitively, you can imagine a higher efficiency in the absence of mechanical friction. You can also imagine virtually zero formation and emission of criteria air pollutants, due to relatively low-temperature and relatively benign electrochemistry. In addition, fuel cells are quiet—a welcomed attribute for deployment as a distributed generator in the midst of where the public resides (homes) and works (industry, office buildings, and hospitals, for example).
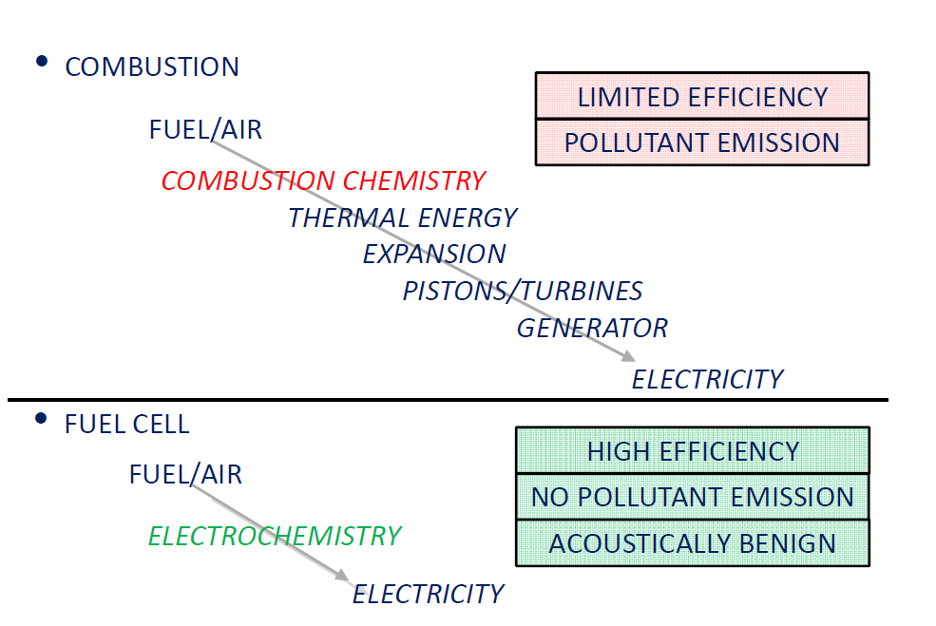
Alternative Fuels
The Energy Policy Act of 1992 defines an alternative fuel as:
- Biodiesel (B100)
- Biodiesel is a renewable fuel that can be manufactured from vegetable oils, animal fats, or recycled cooking grease for use in diesel vehicles.
- Natural gas and liquid fuels domestically produced from natural gas
- Natural gas is a domestically abundant fuel that can have significant cost advantages over gasoline and diesel fuels.
- Propane (liquefied petroleum gas)
- Electricity
- Hydrogen
- Blends of 85% or more of methanol, denatured ethanol, and other alcohols with gasoline or other fuels
- Ethanol is a widely used renewable fuel made from corn and other plant materials. It is blended with gasoline for use in vehicles.
- Methanol, denatured ethanol, and other alcohols
- Coal-derived, domestically produced liquid fuels
- Fuels (other than alcohol) derived from biological materials
- Renewable diesel is a biomass-derived transportation fuel suitable for use in diesel engines.
- P-Series fuels
Transition to Alternative Power Generation for Transportation
The next generation of vehicles is emerging in response to environmental pressures and a goal of fuel independence. The environmental pressures, which include the mitigation of climate change and air quality degradation, require a dramatic reduction in the emission of GHGs and air pollutants from the transportation sector as well as the electric sector. Fuel independence requires removing reliance on the international sourcing of carbon-rich fossil fuels and the associated geopolitics. In response, vehicles of all sizes are transitioning from combustion engines and mechanical drivetrains to alternative vehicles with battery and fuel cell engines and electric drivetrains. The transition began with light-duty vehicles, expanded into medium-duty vehicles, and is now emerging with heavy-duty vehicles including buses. This transition involves a merging of the transportation system with the electricity generation system.
Alternative vehicles encompass fuel cell electric vehicles (FCEVs) and plug-in electric vehicles (PEVs). Examples of PEVs are battery electric vehicles (BEVs) and plug-in fuel cell electric vehicles (PFCEVs).
All of these vehicles have a few key characteristics in common. First, alternative vehicles are designed to operate on fuels that portend (1) a potential of zero emission of both GHG and criteria pollutants and (2) an opportunity to be generated locally and thereby achieve the goal of fuel independence. Second, alternative vehicles have no tailpipe emissions of carbon or criteria pollutants. The GHG and criteria pollutant emissions, if any, come solely from the fuel supply chain, such as the generation of electricity or production of hydrogen. Electricity and hydrogen are the two fuels emerging to power alternative vehicles.
Electricity as a Fuel
For PEVs, the electric grid becomes the source of the fuel. PEVs garner electricity from the home, from the place of work, and in the conduct of business at commercial centers such as big-box stores, shopping centers, and hotels. Referred to as G2V (grid-to-vehicle), extracting energy from the grid adds a new load to the grid. Conversely, PEVs have the potential to provide beneficial attributes to the grid. With what is called V2G (vehicle-to-grid), energy can be extracted from qualified vehicles to serve loads when generating assets are strained.
The existing grid is able to accommodate modest charging events, but as the number of charging events increases (for example, at homes), local transformers may overload and fail. As a result, either upgrades to transformers or controlled charging (that is, smart charging), or both, will be required.
In this process, while the emissions of pollutants from the tailpipes and electric grid are virtually zero and the emission of carbon from the vehicles is zero, the carbon emissions from the electric grid will not be zero with stationary fuel cells (as mentioned above) operating on fossil fuels (for example, natural gas) and biogas. What is required is a zero-carbon fuel.
Hydrogen as a zero-carbon fuel
For FCEVs, hydrogen is the fuel. For PFCEVs, hydrogen is the “longrange” fuel (300 to 400 miles) while electricity is the “short-range” fuel (50 to 150 miles). While the vehicles themselves emit zero carbon, the supply chain of electricity (as noted above) and hydrogen can be major sources of atmospheric carbon if not carefully planned. For example, hydrogen has been traditionally generated in large plants by the steam reformation of natural gas at elevated temperatures. The principal component of natural gas is methane (CH4), with concentrations varying around the world from 70% to over 90%. Other components can be other hydrocarbons (for example, propane and ethane) and inert chemicals such as carbon dioxide and nitrogen.
Today, over 50 million metric tons of hydrogen from steam methane reformation (SMR) are produced annually worldwide, and 11 million metric tons are produced in the United States to support manufacturing (for example, of chemicals, foods, and electronics) and the refining of petroleum to generate gasoline. Notably, the amount of hydrogen needed to fuel 20 million FCEVs in California (today’s population of all vehicles in California) is just 20% more than the hydrogen generated today for the production of gasoline in California. If all the vehicles were PFCEVs, less than 80% would be required. However, SMR hydrogen has an associated emission of CO2. What is required is the generation of renewable hydrogen without the emission of carbon. An initial step in the production of renewable hydrogen is the generation of carbon-neutral biohydrogen using tri-generation for fueling FCEVs and PFCEVs as well as stationary fuel cells. As noted previously, the vast majority of renewable hydrogen is expected to be sourced from the generation of electrolytic zero-carbon hydrogen from otherwise curtailed solar and wind. Not only can electrolytic zero-carbon hydrogen be stored over long periods of time and used in stationary fuel cells as diurnal or seasonal demand requires, it can also be used to fuel FCEVs and PFCEVs.
To use the California example again, systems analyses show that the amount of renewable zero-carbon hydrogen generated by otherwise curtailed renewable resources will be more than ample to fuel FCEVs. While water is also required, fueling all the state’s 20 million vehicles with electrolytic zero-carbon hydrogen would need less than 1% of the daily water flow in the California Aqueduct. If all vehicles were PFCEVs, less than 0.2% would be required.
For dispensing hydrogen to FCEVs, fueling stations are today being deployed at existing gasoline stations. The locations are already zoned for fueling, and the public is familiar with the location as a fueling site. Hydrogen dispensing can be added to an existing island (displacing a gasoline dispenser) or on a newly established fueling island. Over time, gasoline dispensers could be replaced one by one as hydrogen-fueled vehicles displace gasoline-fueled vehicles.
California, again, provides an illustration of the scale of fueling infrastructure that will be required. Approximately 9,800 gasoline stations serve the California population, with multiple stations often sharing the same intersection. However, hydrogen dispensing will not be required at all of the existing gasoline stations. The reasons include the high efficiency of hydrogen vehicles, meaning they can drive farther before refueling than gasoline-powered cars can, and the replacement of competition from the fuel pricing at intersections (often leading to four gasoline stations at an intersection) to the smart phone. For example, it is estimated that a minimum of 1,600 hydrogen stations are needed to fuel a full build-out of FCEVs in 2050. While this number of stations gives drivers a maximum 6-minute access to a hydrogen dispenser, the actual number will likely be larger in order to not overcrowd any one station. If PFCEVs alone were deployed (that is, no FCEVs), the minimum number of stations required statewide would be 93. The larger the percentage of PFCEVs in 2050, the fewer the number of stations over and above 1,600.
In 2019, the number of hydrogen stations in California is approximately 50. They are concentrated at population centers targeted for the introduction of FCEVs by the automobile manufacturers, along with key connector stations (for example, between northern and southern California) and destination stations popular with tourists (for example, Santa Barbara, Lake Tahoe, and Napa Valley).
Zero Emissions Hydrogen Generation Processes
The two technologies commercially available to produce hydrogen with a neutral or zero emission of carbon are steam methane reformation (SMR) using biogas (carbonneutral), and electrolysis powered by otherwise curtailed wind (zero-carbon).
Steam Reformation
Today, most of the hydrogen in the world is generated by SMR as the most cost effective and efficient of all commercial reformation technologies. Efficiencies for centralized natural gas operated SMR plants range from 76 – 81%. SMR operations currently take place mostly on a centralized scale. An example of non-centralized (i.e., “distributed”) hydrogen generation is the SunLine Transit station in Thousand Palms, California. It is likely that more distributed SMR will be introduced into the emerging hydrogen infrastructure since it can take advantage of the existing natural gas infrastructure for wheeling biogas to produce hydrogen on site. Some companies, such as HyRadix, H2Gen, and Ztek, are working on commercializing integrated SMR systems that generate, compress, and dispense hydrogen into vehicles.
Electrolysis
Electrolysis is a method of generating hydrogen from water using an electric current to split water into its two parts: hydrogen and oxygen. The source of the electricity dictates the cost of the process, estimated to be 58% of the price at the pump in one study. Using renewable solar or wind generated electricity that would be otherwise curtailed to power an electrolyzer is an environmentally friendly method to generate zero-carbon hydrogen. Large-scale solar and wind farms can be used for centralized generation of electrolytic hydrogen that can be injected into natural gas pipelines (in the earlier years) and dedicated hydrogen pipelines (in later years), stored from days to seasons, and eventually used to either power fuel cell vehicles or generate electricity through gas turbines or fuel cells. The installation cost of a hydrogen pipeline is 1/3 that of an electrical transmission line that moves the same amount of energy. Hydrogen pipelines are also safer than overhead transmission lines, require less maintenance, and are aesthetically preferred. Electrolysis using the electrical power grid comes at a higher environmental cost. Some studies show that generating hydrogen from grid electrolysis to fuel automobiles yields a net increase of GHG emissions compared to today’s conventional vehicles. However, more recent studies establish that power-to-gas (P2G) technology, which involves the conversion of electrical power into a gaseous energy carrier, is a promising prospect for future energy systems. With P2G, hydrogen can be supplied completely from excess renewable energy, which benefits: 1) balancing the electrical grid with high use of variable, unpredictable renewable power, 2) providing high capacity, long term energy storage for seasonal shifting, and 3) creating a hydrogen supply to promote the use of state-of-art fuel cell vehicles across different transportation sectors, including light, medium, and heavyduty. Additionally, P2G has also been shown to be the most cost-effective approach for longterm energy storage.
The Need for Zero Emissions Buses: A California Case
California Transportation Sector Although public transport by urban bus is generally more environmentally efficient than individual passenger cars, conventional buses are still associated with significant local criteria air pollutant[2] and greenhouse gas emissions[3].
Air pollution associated with criteria pollutants is a significant concern in urban areas such as California’s South Coast Air Basin (SCAB) where heavy-duty vehicles and buses are significant contributors to the total emissions of NOx. While progress has been made in reducing criteria air pollutant emissions from light-duty vehicles, medium-duty and heavy-duty vehicles have been more difficult to address due to the diversity of duty cycles and operational needs encompassed by these sectors. Additionally, powertrains in medium-duty and heavy-duty vehicles have typically relied on diesel fuel in order to satisfy their duty cycles, thereby limiting options for emissions reductions. In addition to criteria air pollutant reductions, many regions of the world have initiated proactive programs to reduce CO2 emissions. California, as an example, has an especially ambitious carbon reduction program. In 2016, total greenhouse gas (GHG) emissions for the State of California were almost 430 million metric tons of CO2 equivalents (MMTCO2e), an overall decrease of 13% from a peak in 2004, and a decrease to 2 MMTCO2e below the 1990 level (the State’s 2020 mandated GHG target).
The transportation sector remains the most significant source of GHG emissions in the State, accounting for 41% of the inventory. Figure 6.5 shows the GHG emission by sector for 2016, and Figure 6.6 presents trends for the transportation sector from 2000 to 2016. Figure 6.6 also shows that heavy-duty vehicles are the second largest contributor to (GHG) emissions behind automobiles and that emissions from the heavy-duty sector started decreasing after 2007, even as diesel sales increased.
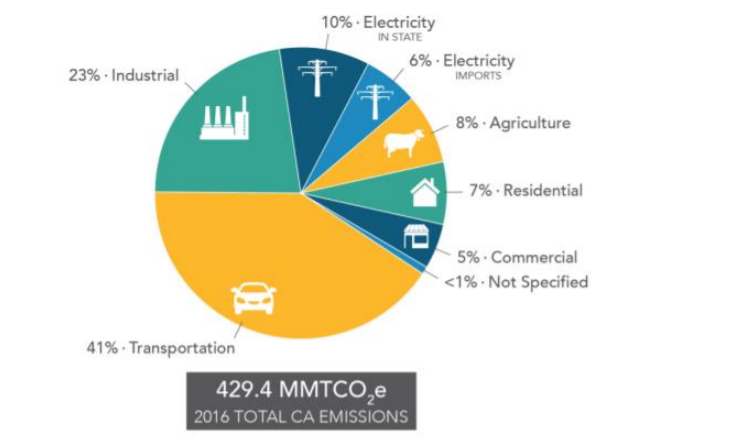
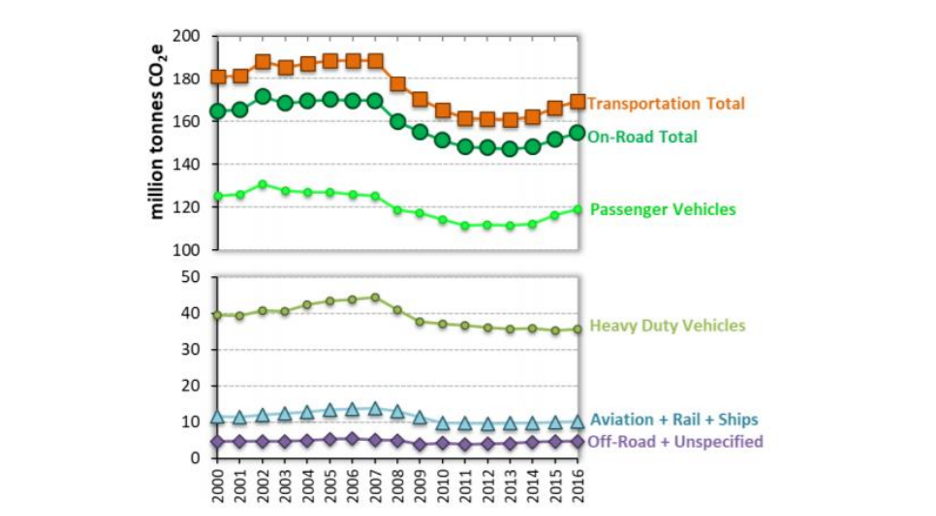
Background Legislation for Urban Buses
In recognition of the need for reduction in greenhouse gas and criteria air pollutant emissions from urban buses, initiatives to deploy zero emission buses into public transit agencies have been promulgated by governmental authorities around the world. For example, during the 2016 International Zero Emission Bus Conference in London, over 23 cities presented plans to incorporate 60% of ZEBs in their fleet by 2025. This includes initiatives such as the C40 Clean Bus Declaration, which stipulates the acquisition of over forty thousand clean buses by 2040. California has been a leader in pollution and GHG emissions reductions for decades. In 2005 Governor Schwarzenegger enacted Executive order S-3-05 which set into motion three GHG emission goals for the State of California in the near and long-term. These goals are to: 1) bring GHG emissions to 2000 levels by 2010, 2) achieve 1990 levels by 2020, and 3) establish GHG emissions levels by 2050 that are 80% below those recorded in 1990. The first two goals were affirmed by the California Legislature in the passage of Assembly Bill 32 (AB 32) in 2016 (the “Global Warming Solutions Act”). While the third goal has not yet been established as a legal mandate, the Legislature passed SB 32 in 2016, which requires by law a 40% reduction from 1990 levels by 2030.
Other legislation, such as Senate Bill 1078, establishes the Renewable Portfolio Standard (RPS), or renewable energy penetration goals for the state. These goals are delineated in Senate Bill 1078 and are updated by Senate Bill 2 with an aim to have a renewable penetration of 20% by 2013, 25% by 2016 and 33% by 2020. These high penetration objectives, along with future increased load from the electrification of the transportation sector, will have complex and dynamic interactions with the electrical grid. These complex interactions and concurrent complementary technology utilization strategies play a crucial role in energy utilization and price regulation. Therefore, to fully leverage these high renewable penetration rates, a sector-wide, California specific approach must be taken when analyzing the future of California’s energy system and build out of complementary infrastructure.
To meet the schedule and reduction targets, a substantial effort has been focused on the transportation sector to accelerate fleet modernization and increase the penetration of clean engine technologies and cleaner fuels. Part of this effort is the “Innovative Clean Transit” initiative from the California Air Resource Board, with the goal of transforming the statewide transit bus fleet by 2040 through phasing-in ZEB purchases. Related to this effort, several transport authorities have stated commitments to transition to a zeroemissions fleet within the next 15 years. These transit agencies, however, will be tasked with complying with these initiatives while still being able to satisfy the travel patterns of customers in their service area. However, no holistic analysis has been conducted to compare the environmental impacts of the overall fuel supply chain needed for the deployment of ZEBs. This type of analysis is essential to: (1) maximize the emission reduction while minimizing resource consumption in the supply chain (e.g., energy and water), and (2) establish criteria that can identify the most effective combination of ZEB technologies based on the characteristics and limitations of the transit agency. Developing strategies to transition urban bus fleets towards low or zero emissions involves selecting between a wide array of emerging public bus powertrain technologies in the context of operational cycle constraints. However, merely deploying battery electric or fuel cell electric buses do not automatically guarantee significant emissions reductions since many sources of emissions may occur outside of the operating or use phase. Therefore, to gain an accurate assessment of how effective the transition to alternate urban bus powertrain technologies can be, accounting for emissions from the full life cycle of these buses must take place.
Heavy Duty Vehicle Electrification
Heavy-Duty Vehicles Electrification of the transportation sector has already begun, with more than 1 million light-duty ZEVs on the road in California as of 2021, consisting of 63% BEVs, 36% plug-in hybrid electric vehicles (PHEVs), and 1% FCEVs. While battery-centric drive trains have dominated the LDV sector, battery powered HDVs have more obstacles because of battery size, battery and vehicle/payload weight, vehicle range, and charging times. A number of HDV vocations (e.g., long-haul, public transit) have long distance travel applications, so their electrified powertrains have to accommodate trips with a long range. Other HDV vocations (e.g., drayage) have shorter ranges and are able to operate satisfactorily with battery power.
Charging infrastructure presents an additional challenge in the heavy-duty space for two main reasons. The first is that charging a high-capacity battery takes much longer than fueling a diesel truck. Some vocations only have one shift a day so can charge overnight, but the rest either must take longer stops for charging or companies must develop a robust cache of battery banks charged at warehouses. The second charging infrastructure challenge is the availability of high-power chargers at the correct times of day. Current public vehicle charging infrastructure includes Level 2 AC chargers and DC fast chargers. While Level 2 chargers can be used for overnight charging of light-, medium-, and some heavy-duty vehicles, they are insufficient to charge the largest HDVs and therefore not a comprehensive option. DC fast chargers can charge light- and medium-duty vehicles quickly, or some HDVs overnight but are not able to charge class 7-8 HDVs in short times. Ultimately, in order to build a sustainable zero-emission HDV future that includes BEVs, an investment in higher power, shorter charge time charging infrastructure around the country is required. Alternative fuels, more specifically FCEVs utilizing hydrogen fuel, also present a sizeefficient solution. They have emerged as a feasible option because hydrogen has a higher gravimetric energy density than gasoline, so it can be compressed and stored on board without imposing as much extra weight as a battery on an already heavyweight system. FCEVs also have comparable fueling times and ranges to internal combustion engine vehicles, making them more competitive than BEVs on that metric in the heavy-duty space. Implementation of FCEVs creates vehicle hydrogen demand (VHD) that motivates hydrogen infrastructure development, allowing for further development of a multi-end use hydrogen economy. Specifically, hydrogen converted to electricity by fuel cells for the electric grid, or electricity hydrogen demand (EHD), is a key part of the diversification of economy-wide uses of hydrogen. However, FCEVs come with their own challenges. Like BEVs with charging infrastructure, hydrogen fueling infrastructure is a large barrier to FCEV penetration. According to the California Fuel Cell Partnership, 8 hydrogen stations in California are dedicated to heavyduty refueling and over 50 additional open and online retail stations. Compared to an estimated more than 10,000 diesel and gasoline stations in the state, that is very early infrastructure for a mode of transportation and severely limits the expansion of FCEV technology at all payloads. In order for FCEVs to be truly zero-emission, the hydrogen production infrastructure must also evolve. As of June 2021, 95% of global hydrogen was gray hydrogen, or hydrogen produced from fossil fuels without the use of any carbon capture and storage (CCS) technology. Gray hydrogen compromises the idea of life cycle zero-carbon transportation, so a transition including FCEVs requires investment in carbon-neutral green hydrogen production methods. Those methods include electrolytic hydrogen using electricity from renewable resources, biogas reformation, and artificial photosynthesis. Once a ZEV fleet mix develops, the shift to electrification will have a notable effect on the grid. BEVs and FCEVs both increase grid demand profiles because BEVs draw power directly from the grid and green hydrogen production for FCEV fueling draws power from the grid for electrolysis albeit utilizing to some extent excess renewable resources.
Electrification will increase peak demand, which is a good indicator of system cost because it defines the maximum capacity at which the grid must be able to comfortably operate [35]. Even though generation does not have to constantly meet peak demand, the transmission system must be able to accommodate it to prevent outages. Fortunately, BEV charging and electrolysis for hydrogen fuel can be considered flexible loads. BEV charging time and rate can be adjusted by the customer manually or smart charging systems can optimize when a network of BEVs charge to minimize cost and strain on the grid. Hydrogen production is even more flexible because the timing of production is not tied to the timing of vehicle fueling; fuel is produced ahead of time, transported to the fueling station, then extracted according to customer needs. Ultimately, the capacity of the electric grid must increase as demand naturally increases over time in addition to the vehicle electrification load. With continued expansion and investment in renewable generation sources, compatible energy storage, and load shifting strategies, peak load can be met and rapid daily ramping managed.
State of the California ZEV Market
California’s Zero Emissions Vehicle (ZEV) market continues to build momentum. In a span of ten years, the market has grown exponentially from a minimal number of total ZEVs in 2009 to over half 600,000 light-duty ZEVs on the roads in California in mid-2019.[4] ZEVs accounted for nearly eight percent of new light-duty vehicle sales in 2018, which represents a growth in market share of almost 40 percent compared to 2017. Additionally, there are 47 zero-emission light-duty cars and trucks offered for sale or lease in California with more planned in the coming years. While the majority of the ZEVs sold have been Plug-in Hybrid Electric Vehicle (PHEVs) and Battery Electric Vehicle (BEVs), the younger light-duty fuel cell electric vehicle (FCEV) market is gaining momentum growing from fewer than 100 a decade ago to approximately 6,000 on California’s roads by mid-2019. The heavyduty ZEV market is also growing rapidly as ZEV technology transfers from light-duty and smaller heavy-duty ZEV applications, with over 100 models commercially available today and many major manufacturers announcing plans for future commercialization of battery-electric and hydrogen fuel cell electric trucks and buses.
Refueling infrastructure is needed to power the vehicles and support the ZEV market. As of December 2019, California has 22,233 electric vehicle charging outlets, including 3,355 direct current fast chargers (DCFCs), at over 5,674 public stations throughout the State and 41 public retail hydrogen stations located in the major metropolitan areas compared to virtually none a decade ago. The State’s goal is to have 1.5 million ZEVs on the road, 250,000 charging outlets, including 10,000 DCFC, and 200 hydrogen stations by 2025 as well as 5 million ZEVs by 2030. The magnitude and speed of effort needed to achieve these goals is unprecedented.
Historical Alternative Fuels and Vehicles Incentive Programs in California
Air Quality Improvement Program (AQIP)
The Air Quality Improvement Program (AQIP), established by the California Alternative and Renewable Fuel, Vehicle Technology, Clean Air, and Carbon Reduction Act of 2007 (Assembly Bill (AB) 118, Statutes of 2007, Chapter 750), was a voluntary incentive program administered by CARB to fund clean vehicle and equipment projects, research on biofuels production and the air quality impacts of alternative fuels, and workforce training. The AQIP Guidelines and annual funding plans guided CARB’s implementation of the AQIP.
- Hybrid Truck and Bus Voucher Program
- AQIP background, working group information and regulatory text (available by request)
- Program administrator page with list of eligible vehicles / application forms
- Zero-Emission Vehicle and Plug-In Hybrid Light-Duty Vehicle Rebate Project
- AQIP background, working group information and regulatory text (available by request)
- Program administrator page with list of eligible vehicles / application forms (Clean Vehicle Rebate Project)
Carpool / HOV Lane Access
California law allows single-occupant use of High Occupancy Vehicle (HOVs) lanes by certain qualifying clean alternative fuel vehicles. Use of these lanes with a single occupant requires a Clean Air Vehicle Sticker issued by the California Department of Motor Vehicles (DMV).
Alternative Fuel Incentive Program (AFIP) 2007-2009
The Alternative Fuel Incentive Program (AFIP), established by Assembly Bill (AB) 1811, required CARB to develop a joint plan with the California Energy Commission to spend $25 million for the purposes of incentivizing the use and production of alternative fuels. This program has ended.
ZIP I, ZIP II and Fleet ZIP 2001-2004
The Zero Emission Vehicle Incentive Programs (ZIP I, ZIP II and Fleet ZIP) were administered by CARB in conjunction with the State Energy Resources Conservation and Development Commission (California Energy Commission or CEC).
Lawn and Garden Equipment Replacement Project
The purpose of the Air Quality Improvement Program (AQIP) Lawn and Garden Equipment Replacement (LGER) Project was to encourage further development and deployment of cordless zero-emission lawn and garden equipment. The availability of incentive funding helped bring a variety of residential and commercial cordless zero-emission equipment to the market.
Zero-Emission All-Terrain Agricultural Work Vehicle Rebate Project
The $1.1 million Zero-Emission Agricultural UTV Rebate Project accelerated the use of zero-emission work vehicles for use in California agricultural operations, by providing rebates for the purchase of new, eligible all terrain and utility vehicles on a first come, first serve basis.
Key Takeaways
- Battery Electric Vehicles (BEVs) are sufficient and, with advances in battery technology, the energy density will dramatically increase, the charging time will dramatically decrease, and the weight will dramatically decrease to provide the range, fueling time, and size provided historically by petroleum-fueled internal combustion vehicles.
- While BEVs have a role, FCEVs and PFCEVs are needed to provide the range and refueling time to which the public is accustomed with conventional gasoline and diesel internal combustion vehicles.
- Fuel Cell technology is also suitable for medium-duty vehicles (such as delivery trucks) and for heavy-duty vehicles (that is, buses and large trucks) where BEV technology is limited or insufficient. FC technology is applicable as well for off-road construction vehicles, locomotives, and ships.
Self-Test
Glossary: Key Terms
Battery electric vehicles (BEVs): All-electric vehicles, also called battery electric vehicles, have a battery that is charged by plugging the vehicle in to charging equipment. These vehicles always operate in all-electric mode and have typical driving ranges from 150 to 400 miles.
Fuel cell technology: A fuel cell uses the chemical energy of hydrogen or other fuels to cleanly and efficiently produce electricity
Fuel cell electric vehicles (FCEVs): FCEVs use a propulsion system similar to that of electric vehicles, where energy stored as hydrogen is converted to electricity by the fuel cell.
Plug-in electric vehicles (PEVs): A plug-in electric vehicle (PEV) is any road vehicle that can utilize an external source of electricity (such as a wall socket that connects to the power grid) to store electrical energy within its onboard rechargeable battery packs, to power an electric motor, and help propelling the wheels.
Plug-in fuel cell electric vehicles (PFCEVs): Plug-in fuel cell electric vehicles (PFCEVs) combine features of battery electric vehicles (BEVs) and fuel cell electric vehicles (FCEVs). This vehicles are a type of plug-in hybrid electric vehicle where instead of an internal combustion engine a hydrogen gerating fuel-cell is used.
Media Attributions
- Figure 6.1: Los Angeles 1943: Degraded Unraban Air Quality by Ramanathan et al is licensed under CC BY-NC-SA 4.0
- Figure 6.2: Combustion by Ramanathan is licensed under CC BY-NC-SA 4.0
- Figure 6.3: Combustion Impacts by Ramanathan et al is licensed under CC BY-NC-SA 4.0
- Figure 6.4: Power Generation Options by Ramanathan is licensed under CC BY-NC-SA 4.0
- Figure 6.5: Greenhouse Gas Emissions by Sector for 2016 by Analy Castillo is licensed under CC BY-NC-SA 4.0
- Figure 6.6: Greenhouse Gas Emissions for the Transportation Sector in California by Analy Casillo is licensed under CC BY-NC-SA 4.0
References
- Ramanathan, V., Aines, R., Auffhammer, M., Barth, M., Cole, J., Forman, F., et al. (2019). Bending the Curve: Climate Change Solutions. Location: Regents of the University of California. Editor: V. Ramanathan. Co-Editors: Adam Millard-Ball; Michelle Niemann; Scott Friese. Book published by the Regents of the Univ of California. Retrieved from https://escholarship.org/uc/item/6kr8p5rq. 815pp.under (CC BY-NC-SA 4.0)
Attributions
- Bending the Curve: Climate Change Solutions by Ramanathan, V., Aines, R., Auffhammer, M., Barth, M., Cole, J., Forman, F., et al. is licensed under CC BY-NC-SA 4.0
- Technology Mix Optimization for Zero-Emission Fleets Adopting a Multi-Criteria Decision Analysis within a Life Cycle Assessment Framework by Castillo, Analy is licensed under CC BY-NC-SA 4.0
- Viable Pathways in the Energy Sector to Meet California Climate Goals with an Emphasis on Heavy-Duty Transportation and Refinery Emissions by Houck, Margaret is licensed under CC BY-NC-SA 4.0
- Ramifications of combustion exhaust were observed centuries before, an example of which is “fumifugium” (Evelyn 1661). ↵
- Air pollution contributes to a wide variety of adverse health effects. The U.S. Environmental Protection Agency has established national ambient air quality standards (NAAQS) for six of the most common air pollutants— carbon monoxide, lead, ground-level ozone, particulate matter, nitrogen dioxide, and sulfur dioxide. ↵
- Gases that absorb infrared radiation and trap heat in the atmosphere are called greenhouse gases. ↵
- Veloz, 2019. “Sales Dashboard.” Last updated: August 6, 2019. https://www.veloz.org/sales-dashboard/. Accessed August 15, 2019. ↵
A fuel cell uses the chemical energy of hydrogen or other fuels to cleanly and efficiently produce electricity
FCEVs use a propulsion system similar to that of electric vehicles, where energy stored as hydrogen is converted to electricity by the fuel cell.
All-electric vehicles, also called battery electric vehicles, have a battery that is charged by plugging the vehicle in to charging equipment. These vehicles always operate in all-electric mode and have typical driving ranges from 150 to 400 miles.
Plug-in fuel cell electric vehicles (PFCEVs) combine features of battery electric vehicles (BEVs) and fuel cell electric vehicles (FCEVs). This vehicles are a type of plug-in hybrid electric vehicle where instead of an internal combustion engine a hydrogen gerating fuel-cell is used.